Lior Artzia - Margulis Prize applicant
Tali Dadoshb - was involved in the design of the project and co-performed the superresolution and correlative imaging
Elad Milrotb helped prepare samples and SEM imaging, co-performed correlative imaging.
Sarah Moraïsa helped calibrate immunolabeling of the cells.
Smadar Levin-Zaidmanb helped perform sample preparations and TEM imaging experiments
Ely Moraga - participated in the project design
Edward A. Bayera – the principal investigator
aDepartment of Biomolecular Sciences, The Weizmann Institute of Science, Rehovot, Israel bDepartment of Chemical Research Support, The Weizmann Institute of Science, Rehovot, Israel
The plant cell wall is the most abundant carbon source on earth and comprises a variety of polysaccharides, mainly cellulose, which is highly resistant to enzymatic degradation (1). Cost-effective strategies to break down cellulose will provide soluble sugars that can be fermented to biofuels, thus alleviating our reliance on fossil fuels (2).
Cellulosomes are large multienzyme complexes produced by certain anaerobic bacteria to degrade plant cell wall polysaccharides, including cellulose. The cellulosome is composed of a large set of hydrolytic enzymes organized by non-catalytic, structural proteins called scaffoldins into a highly efficient degradative machine (3, 4). Clostridium clariflavum is an anaerobic thermophile, that produces the largest assembled cellulosome complex known in nature, composed of 185 enzymatic and structural subunits (5). This 4-tiered cell surface-attached complex is composed of a cell-anchoring scaffoldin (ScaC), 4 adaptor scaffoldins (ScaB) that allow multiplication of the enzymes in the complex, and 20 primary scaffoldins (ScaA), that interact with 160 enzymatic subunits (Figure 1A). This architectural flexibility multiplies the cellulosomal enzyme compositions in this bacterium.
To investigate these surface-associated cellulosomes, we used monoclonal and polyclonal antibodies against 4 representative cellulosome components, one from each tier (Figure 1A), to define their relative subcellular localization. By using superresolution microscopy (STORM), we observed co-localization among the successive cellulosomal components. The cells were labeled by monoclonal and polyclonal antibody pairs to study their relative localization in the complex (Figure 1C-F). By taking this approach, we were able to localize ScaC at the most proximal level of the complex, relative to the cell surface. ScaB is situated between ScaC and ScaA, ScaA is distal to ScaB and the enzymatic subunits were located at the most external layer of the complexes. This is the first time that the hierarchical organization of cellulosomes could be presented visually in vivo. By quantifying the clusters of ScaC on the cell surface of cells grown on different soluble (e.g., cellobiose) and recalcitrant insoluble (cellulose and wheat straw) carbon sources, we revealed large variations in the number of surface-associated cellulosomes depending on the carbon source used for cell growth, suggesting novel control mechanism(s) for cellulosome surface display and assembly.
In addition, we investigated the morphological localization of cellulosomes. Cell-surface protuberances had previously been observed SEM and TEM. We showed by TEM that cellulosome components are localized exclusively on the cell surface (Figure 2). SEM revealed a similar pattern of protuberances, as we observed by immunolabeling of cellulosomes, and correlative STORM-SEM (CLEM) showed that cellulosomes are specifically localized in these protuberances, indicating that the bacterium creates specialized cell-surface compartments for cellulosomes (Figure 3).
The results of this study provide definitive experimental proof of the presumed architecture of a cellulosome system, which had previously been based on the logical assessment of the biochemically determined interactions of the cellulosome component parts. Our results thus provide conclusive insight into the spatial organization of cellulosomes on the bacterial cell surface and reveal that the bacterial cell requires closely attached surface cellulosomes to break down cellulosic substrates. This information is crucial for designing efficient plant cell wall deconstruction processes, whereby the bacterial cell, together with the secreted proteins, plays an important role in polysaccharide degradation (6).
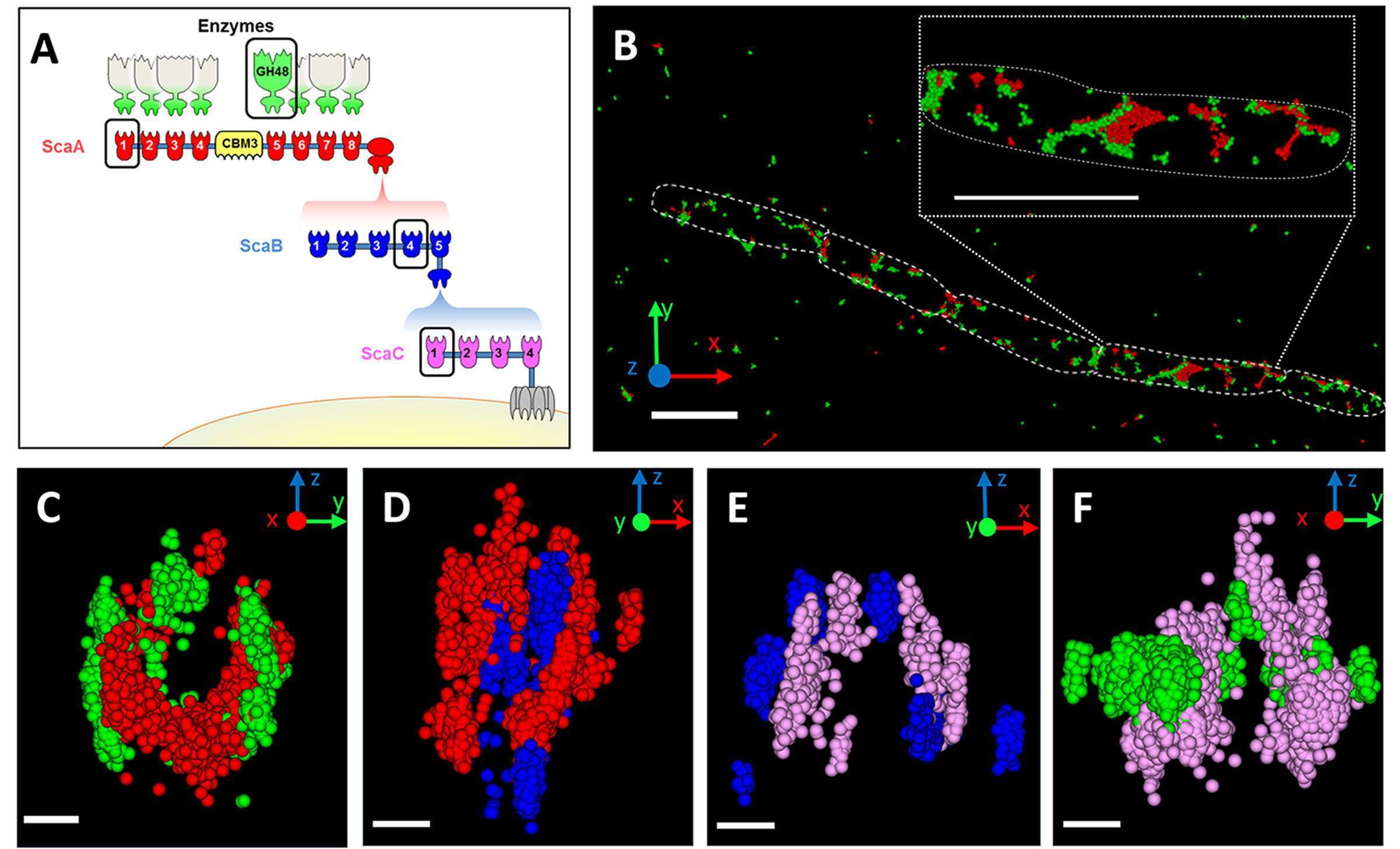
Figure 1: Hierarchical organization of cell-surface cellulosome components. (A) Schematic illustration of the major cellulosome system of C. clariflavum. Color-coded cohesins (Coh) and enzyme (GH48) components circled in black were immunolabeled during experiments. (B) Representative 3D STORM image of cellobiose-grown cells labeled with anti-GH48 (green) and anti-CohA (red). Scale bar, 2 µm. (C to F) Representative cross-sectioned STORM images of selected bacterial cells immunolabeled with pairs of antibodies (scale bars, 300 µm). A portion of each bacterium was sectioned and rotated 90° for viewing through its long axis. (C) Anti-GH48 (green) and anti-CohA (red); (D) anti-CohA (red) and anti-CohB (blue); (E) anti-CohB (blue) and anti-CohC (pink); (F) anti-CohC (pink) and anti-GH48 (green).
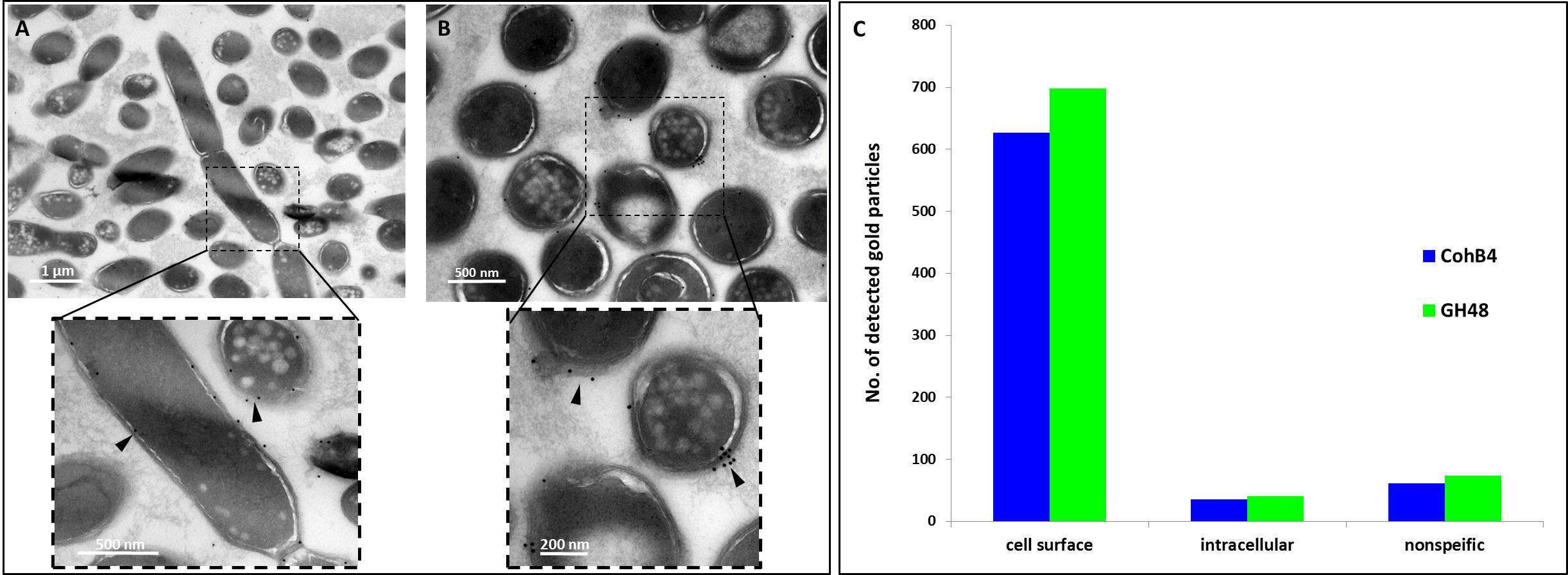
Figure 2: TEM immunolabeling of C. clariflavum. Cells were immunolabeled (arrows) with gold-derivatized anti-CohB4 (A) or anti-GH48 (B). (C) Cellulosome particles are exclusively localized to the cell surface (cells labeled by anti-CohB4 n=217 and by anti-GH48 n=269).
Figure 3: CLEM images of C. clariflavum. (A and B) Cellobiose-grown cells were immunolabeled with anti-GH48 (green) and anti-CohC (pink). (A) Scale bars, 1 µm (200 nm in each inset). (B) Scale bars, 2 µm (200 nm in each inset). (C and D) Wheat straw-grown cell, immunolabeled with anti-CohA and anti-CohB. (C) Labeling is colored according to the specific probes: anti-CohA, red; anti-CohB, blue. (D) The depth of labeling is presented for the two combined probes, color-coded from yellow (shallow labeling) to dark red (deep labeling, closer to the slide). Scale bars, 1 µm (200 nm in each inset in panel C).
References
- Gilbert HJ. 2010. The biochemistry and structural biology of plant cell wall deconstruction. Plant Physiol 153:444–55.
- Bayer EA, Lamed R. 1992. The cellulose paradox: pollutant par excellence and/or a reclaimable natural resource? Biodegradation 3:171–88.
- Bayer EA, Shoham Y, Lamed R. 2013. Lignocellulose-decomposing bacteria and their enzyme systems, p. 215–266. In Rosenberg, E, DeLong, EF, Lory, S, Stackebrandt, E, Thompson, F (eds.), The Prokaryotes. Springer Berlin Heidelberg, Berlin, Heidelberg.
- Artzi L, Bayer EA, Moraïs S. 2016. Cellulosomes: bacterial nanomachines for dismantling plant polysaccharides. Nat Rev Microbiol 15:83–95.
- Artzi L, Dassa B, Borovok I, Shamshoum M, Lamed R, Bayer EA. 2014. Cellulosomics of the cellulolytic thermophile Clostridium clariflavum. Biotechnol Biofuels 7:100.
- Artzi L, Dadosh T, Milrot E, Moraïs S, Levin-Zaidman S, Morag E, Bayer EA. 2018. Colocalization and disposition of cellulosomes in Clostridium clariflavum as revealed by correlative superresolution imaging. mBio 9:e00012-18.