Radiative heating dominates forebody thermal loads on re-entry capsules travelling at super-orbital speeds, and was recently identified as a major component in afterbody heating as well[1]. With a strong shock wave formed around the forebody, the shock layer is highly populated with electronically-excited nitrogen and oxygen atoms, which continue to radiate as the flow expands around the afterbody. Although subjected to less severe heating, sizing the afterbody heat shield is a significant design challenge, which requires a large structural safety factor[2], due to the uncertainties associated with the rapid expansion of the flow from the forebody to the leeward surfaces. New experimental data is required before theoretical models of the non-equilibrium recombination processes, which occur in the expanding flow, can be validated, and more accurate predictions of the base heating loads can be made by spacecraft designers. As a first step, a proof-of-concept experimental campaign was launched to interrogate the radiation from a rapidly expanding flow of air with a total enthalpy of 74.5MJ/kg in the X2 expansion tunnel at the University of Queensland. A simple geometry was chosen comprising a 54° 2D compression ramp followed by a Prandtl-Meyer (P-M) expansion fan, as is illustrated in Figure 1. Emission spectroscopy was employed and spectral measurements spanning the range from ultraviolet (UV) to near-infrared (NIR) were taken behind the shock wave and in the expansion fan. Spectra were acquired at various distances from the model surface and from the centre of the P-M expansion fan, so that the effects of the length scales associated with the non-equilibrium expanding streamlines could be studied. Streamlines passing close to the corner (such as Streamline 1 in Figure 1) were expanded very rapidly, and approximated ‘frozen’ flow, whilst those traversing the outer region of the expansion fan (such as Streamline 2 in Figure 1) would be expanded more gradually over a longer physical distance and would experience different levels of non-equilibrium. Example NIR spectra taken at 10mm above the model shoulder are presented in Figure 2. In addition, a Thorlabs FBH780-10 bandpass filter was coupled to an ICCD camera to obtain images of the 777nm oxygen triplet development. These images were calibrated with an integrating sphere to generate spatial distribution of the spectral radiance, as is exhibited in Figure 3. Both qualitative and quantitative comparisons were made between the measurements and two-temperature CFD simulations with finite-rate 11-species air reactions. Analyses and discussions will be presented and plans for future experiments will be discussed in the full paper.
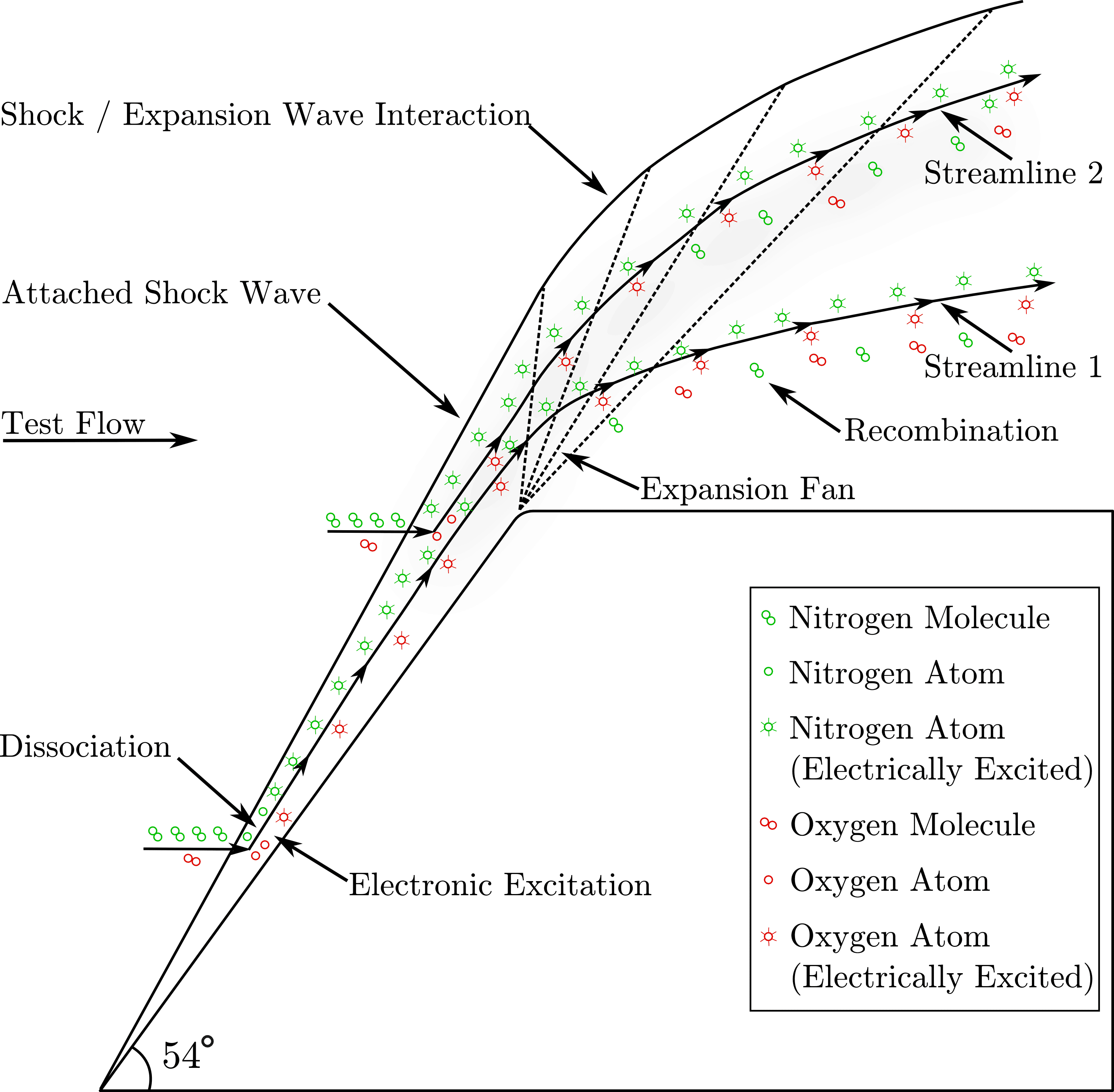
Figure 1: Schematic of the model as well as the physical processes involved
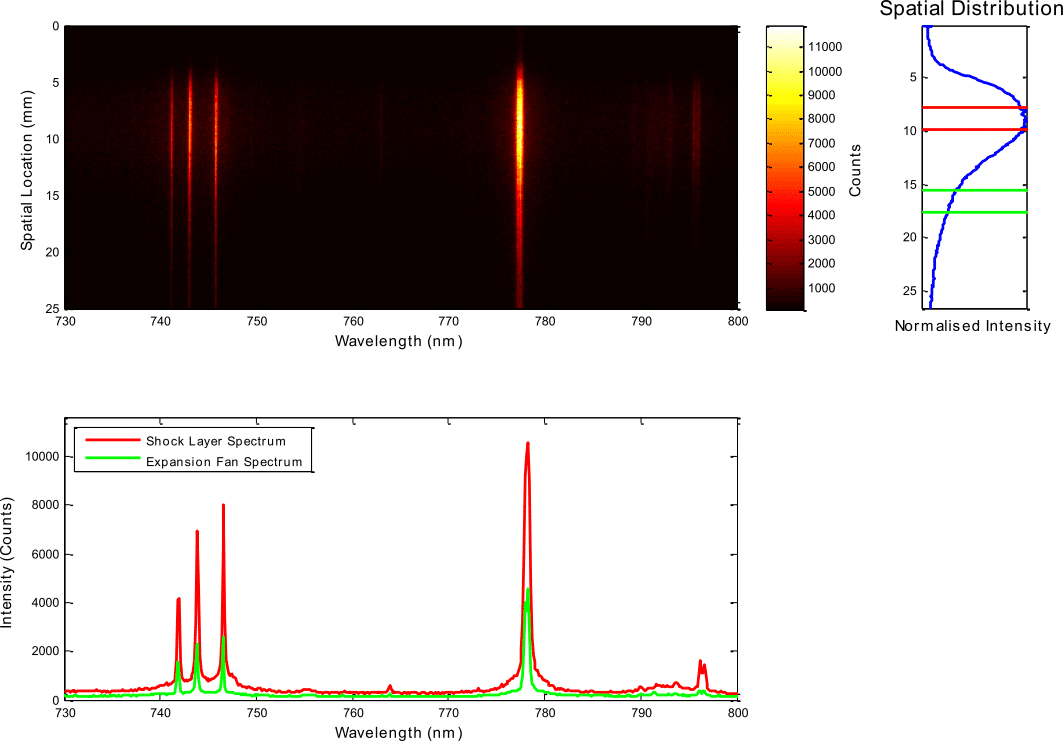
Figure 2: NIR spectra at 10mm above model top surface

Figure 3: Spectral radiance distribution of 777nm oxygen triplet
References
[1] Christopher O. Johnston and Aaron M. Brandis. “Features of Afterbody Radiative Heating for Earth Entry”. In: 11th AIAA/ASME Joint Thermophysics and Heat Transfer Conference. AIAA Aviation. American Institute of Aeronautics and Astronautics, June 13, 2014.
[2] Michael J. Wright, Frank S. Milos, and Phillippe Tran. “Afterbody Aeroheating Flight Data for Planetary Probe Thermal Protection System Design”. In: Journal of Spacecraft and Rockets 43.5 (Sept. 2006), pp. 929–943.